Main navigation (level 2) (loaded programmatically)
Energy offers comfort and economic development for those who have access to it. It enables a high quality of life and if properly managed, makes humans healthy, prosperous and free. In fact, it enables our access to clean water and abundant food as well. However, energy choices are inherently about underlying trade-offs, as energy done the wrong way can inhibit economic growth, impinge on the environment and undermine security as highlighted by the Russian war in Ukraine. To limit global temperature increase to maximum 1.5 °C above the pre-industrial level as agreed in the Paris Agreement, carbon neutrality should be reached by 2050. Reaching that goal implies that by 2050, no net carbon emissions should occur anymore and that all carbon emitted into the air, will have to be compensated by CO2 sinks, either natural or artificial. As argued previously (Mertens et al.1), the prioritisation for action is as follows:
- First and foremost, we must (continue to) increase the energy efficiency of all our activities and processes and thus consume less energy. Energy efficiency remains a priority. Even though it is often considered that the low-hanging fruit of energy efficiency has been harvested, especially there remains tremendous improvement margin for it.
- We should increase the share of renewable electricity production and use it to electrify as many processes as possible. This effort must go far beyond electrical transportation. It should include building heating and cooling, as well as many industrial processes and seawater desalination.
- For processes where high energy density is crucial or for the chemical industry where hydrocarbons are needed as a feedstock or for the storage of energy over longer time periods, the need for molecules will remain important. Hydrogen production is the most obvious e-molecule since it can be synthesised from renewable electricity and water, either locally or anywhere in the world where cheap renewable electricity and water are available. As discussed in detail in Mertens et al1 (2020), due to its low volumetric energy density and the challenges related to its storage and transport, using this green hydrogen in combination with CO2 or N2 and convert it to high energy dense molecules (e.g. methane, methanol, ammonia, jet fuel, …) will be crucial for long-distance marine and aviation transportation, high-temperature heat in the industry, chemicals, long-distance transport of renewable energy and long-term energy storage.
On our path to carbon neutrality, energy efficiency and electrification remain of utmost importance and it is why we refer to them as number 1 and 2 in the action prioritisation list above. However, it is clear that there is a need for sustainable molecules for applications that require high energy density, e.g., shipping, aviation, high temperature processes, feedstock for chemistry, high-temperature heat, among others, or for the transport and storage of renewable energy over longer distances and time periods. These sustainable molecules include several types: biofuels based on wastes and residues (such as biomethane, bioethanol, biodiesel, …) and electricity-based e-fuels (such as hydrogen or hydrogen-derived molecules made from electricity). Biofuels are useful drop-in alternatives, but are limited on a global scale, because of competition with land use for food. Green hydrogen, that is hydrogen made from water electrolysis powered by renewable electricity, is a prominent e-molecule that captures a lot of headlines and the attention of policymakers, but is challenging to transport and store.
Figure 1 summarizes the volumetric energy issue by illustrating the volumes needed to store 10 kWh of energy using different energy vectors: battery electricity, hydrogen (liquid or at pressure), liquid ammonia (NH3), LNG (liquefied natural gas), and petrol.
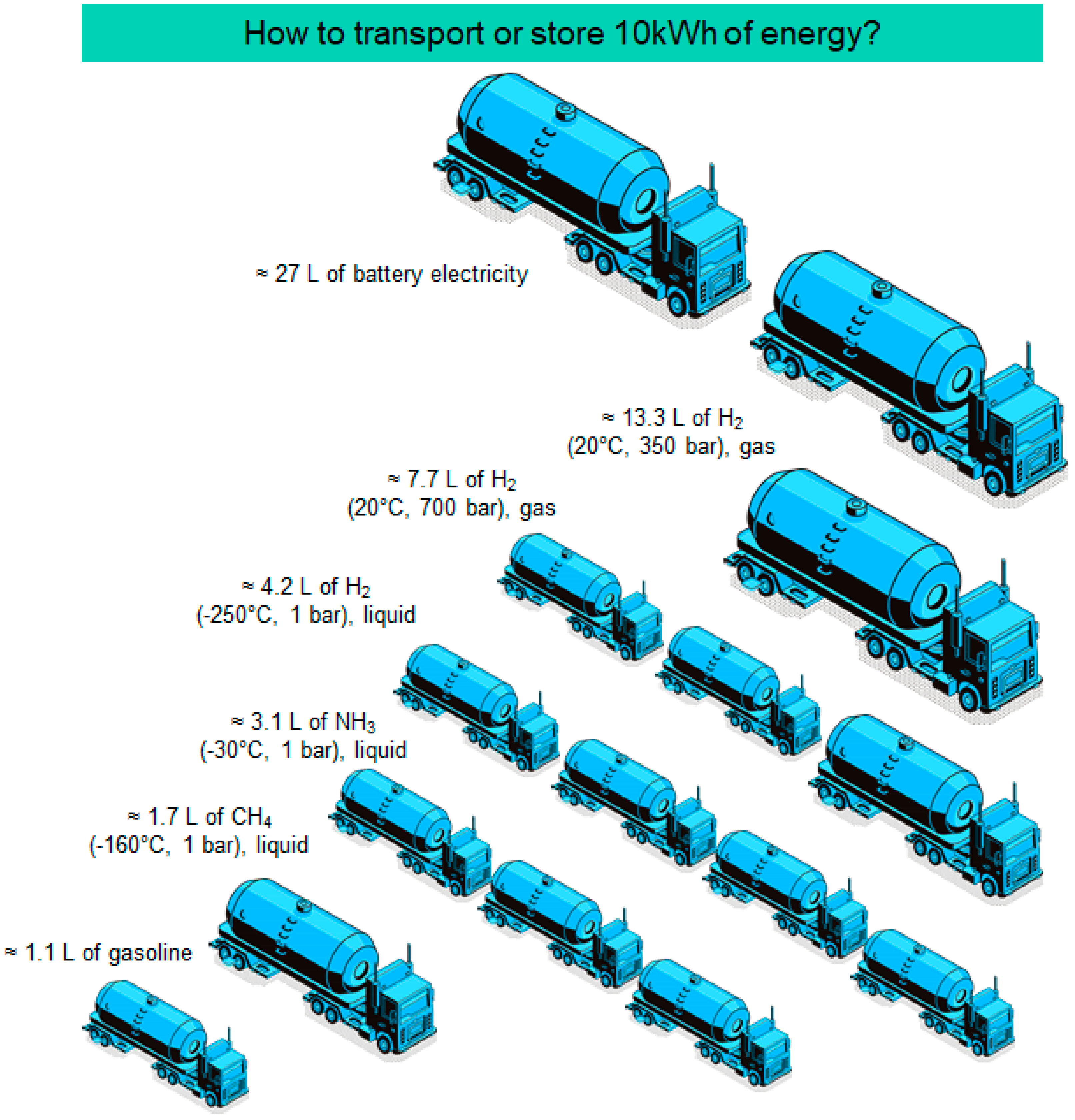
This figure is complemented by Table 1 giving the numbers for the volumetric energy density and the gravimetric energy density or specific energy of the different energy vectors.
Given the physical properties depicted in figure 1 and Table 1, wherever possible, the direct use of hydrogen at the production site is the preferred option.
However, in many cases this will not be possible since the production of hydrogen will mainly be
in areas with abundant cheap renewable electricity that do not necessarily coincide with large industrial areas (with steel, cement, chemical, glass facilities, etc.) where enormous energy demands exist. In that case, just as we do today, transport of energy will be needed and as illustrated in figure 1, this is best done using a hydrogen derived molecule rather than hydrogen itself. The same is true for the storage of renewable energy over long time periods such as seasons for example where again hydrogen is a challenging option and its derived molecules are much more energy dense.
Today, almost all approaches to synthesize renewable fuels are more expensive in comparison to their fossil alternatives [3]. If the extrapolation of the past exponentially reducing costs of photovoltaics (PV) and wind electricity continues in future, optimistic scenarios show competitive costs for renewable hydrogen production already in 2027 and for renewable synthetic fuels soon after [4]. Studies show that the cost of the renewable fuel is dominated by the cost of this green hydrogen production, i.e., the operational expenditure (OPEX) related to the renewable electricity production and the capital expenditure (CAPEX) of the electrolyzer, which is still expensive today.
For sure is that, due to efficiency losses in the (atmospheric) CO2 capture and conversion steps, the production of renewable molecules will drastically increase the overall demand for renewable energy. This however should not exclude carbon-based carriers from the path to zero carbon. Therefore a “Synthetic Hydrocarbon Economy” should be considered next to a pure “Hydrogen Economy”.
Moreover, the use of hydrogen in the replacement of fossil fuels sets large challenges in terms of equipment revamping and technology developments.
The production of these e-molecules will require huge amounts of renewable energy, in particular to produce green hydrogen. Many regions, like western Europe with limited access to renewable energy relative to the anticipated overall energy demand, may remain dependent on imports 3 from countries where access to low-cost and abundant renewable resources is feasible. High energy density e-molecules turn out to be efficient energy carriers over long distances (e.g. methanol, ammonia, methane, jet fuel, …). Another advantage of e-fuels made from hydrogen is that they can be easily used as a drop-in fuel into the existing energy infrastructure, with an ultimate aim to maximise the value of the existing assets and minimise total cost. Consequently, this infrastructure will be carrying renewable molecules instead of fossil ones. However, when available and technically feasible, direct electrification options are typically lower in cost and higher in overall efficiency, which cannot be compensated by prolongation of existing infrastructure use4,5.
IEA’s ETP 20206 report estimates that 38% of the emission reduction that is needed will come from making our electricity fully renewable. This potential improvement is not sufficient. Another 40% of the effort will have to come from reducing emissions from industrial processes, and the IEA concludes that ‘CCUS’ (Carbon Capture Utilisation and Storage) will play a major role in this part. It has to be emphasised that CCUS is a misleading term, as the impact and business cases for CCU and CCS are fundamentally different and grouping both disjunct approaches does not reflect the reality of these two approaches7,8. IEA is not the only one as reports from the German Energy Agency3 , LUT University4, Stanford University9, and others drew similar conclusions that fuels will be a necessary part of a swift, affordable and reliable trajectory to net-zero carbon emissions. In the supplementary information section A, the case study for Belgium is given as example to highlight that molecules will be needed to reach carbon neutrality.
Apart from renewable electricity, a source of carbon, hydrogen, oxygen, or nitrogen is also required to fabricate synthesised molecules such as ammonia (NH3), methane (CH4), methanol (CH3OH), dimethyl ether (CH3OCH3), or formic acid (HCOOH). These sources are usually water or the atmosphere. Carbon capture from point sources is a mature technology to separate CO2 from flue gases. Cryogenic air separation is a mature electrified process to separate O2 and N2 from the air. Direct Air Capture (DAC) is an emerging technology to concentrate CO2 from the air10. DAC has many opportunities and challenges that will be further discussed in the paper.
There exist several myths and beliefs around the topic of e-molecules and this paper aims at demystifying some of them, more info in Mertens et al.11
Jan Mertens
Chief Science Officer Engie
Professor UGent
1 Mertens, J., R. Belmans en M. Webber, 2020. Why the carbon neutral transition will imply the use of lots of carbon. C-Journal of Carbon Research, 6 (39), 1-8
2 Detz, R.J.; Reek, J.N.H. en van der Zwaan, B.C.C. The future of solar fuels: When could they become competitive? Energy Environ. Sci., 2018, 11, 1653–1669.
3 Ram M., T. Galimova, D. Bogdanov, M. Fasihi, A. Gulagi, C. Breyer, M. Micheli and K. Crone, 2020. Powerfuels in a Renewable Energy World - Global volumes, costs, and trading 2030 to 2050. LUT University and Deutsche Energie-Agentur GmbH (dena). Lappeenranta, Berlin
4 Bogdanov, D., M. Ram, A. Aghahosseini, A. Gulagi, A.S., Oyewo, M. Child, U. Caldera, K. Sadovskaia, J. Farfan, L. De Souza Noel Simas Barbosa, M. Fasihi, S. Khalili, T. and C. Breyer. 2021. Low-cost renewable electricity as the key driver of the global energy transition towards sustainability. Volume 227, 120467
5 Ueckerdt, F., C. Bauer, A. Dirnaichner, J. Everall, R. Sacchi and G. Luderer, 2021. Potential and risks of hydrogen-based e-fuels in climate change mitigation. Nat. Clim. Chang., 11, 384–393
6 IEA, 2020. Energy Technology Perspectives 2020. https://www.iea.org/reports/energy-technology-perspectives-2020
7 Bruhn, T., H, Naims and B. Olfe-Kräutlein 2016. Separating the debate on CO2 utilisation from carbon capture and storage. Environmental Science & Policy, 38-43
8 Breyer, B., M. Fasihi, C. Bajamundi and F. Creutzig, 2019. Direct Air Capture of CO2: A Key Technology for Ambitious Climate Change Mitigation. Joule, 9 , 2053-2057
9 Jacobson, M.Z., M. A. Delucchi, M. A. Cameron, S. J. Coughlin, C.A. Hay, I. Priya, Manogaran, Y. Shu and A-K von Krauland, 2020. Impacts of Green New Deal Energy Plans on Grid Stability, Costs, Jobs, Health, and Climate in 143 Countries. One Earth, 1, Pages 10
10 Fasihi, M., O. Efimova and C. Breyer, 2019. Techno-economic assessment of CO2 direct air capture plants. Journal of Cleaner Production, 224, 957-980
11 Mertens, J., C. Breyer, K. Arning, A. Bardow, R. Belmans, A. Dibenedetto, S. Erkman, J. Gripekoven, G. Léonard, S. Nizou, D. Pant, A.S. Reis-Machado, P. Styring, J. Vente, M. Webber and C. J. Sapart, 2022. Carbon Capture and Utilisation: More than hiding CO2 for some time, Joule, https://doi.org/10.1016/j.joule.2023.01.005